
Desperate remedies
When radioactivity was discovered at the end of the 19th century, it surprised scientists. Radioactive decay could transmute one element to another – a conversion previously thought possible only in the dreams of alchemists. In the process, mysterious beams of energy were emitted from the source material, beams that were at first mistaken for a type of light, but soon revealed to be something else altogether. By examining the extent to which these radioactive emissions were able to penetrate other materials, scientists realised that there were different types of decay. Alpha decay resulted in emissions that could be blocked by a thin sheet of paper, whereas gamma decay resulted in emissions that could not be stopped by anything short of a very thick layer of lead.
In terms of penetrating power, a third type of decay, beta decay (or β-decay), was neither exceptionally weak nor exceptionally strong: these emissions could penetrate paper but not sheets of metal. By the early 1900s, scientists knew that beta decay was a process that emitted electrons. But experiments on these emitted electrons raised a mystery of their own. Whereas other types of radioactive emissions always had a discrete amount of energy, which was characteristic of the source material, the electrons that were emitted during β-decay took a continuous range of energy values. This result, which was called the continuous β-spectrum, meant that if you took two electrons that had been emitted from the very same source material during β-decay, one of these electrons sometimes had less energy than the other, and the difference in the amount of energy between the two electrons varied.
Where did the energy that was present in one case, and missing in the other case, go? This was a crucial question because, if it could not be answered, β-decay would involve a violation of the conservation of energy. Some of the greatest experimental physicists of the time revisited this surprising result again and again, but more than 15 years after the continuous β-spectrum was first observed, it still wasn’t clear what explained it.
In early December 1930, Wolfgang Pauli penned an open letter to a group of nuclear physicists who were gathering for a meeting in Tübingen in Germany. Pauli was just 30 years old but already well known for his work on relativity and foundations of quantum theory (including the Pauli exclusion principle). He had been puzzling over the continuous β-spectrum for several years. ‘Dear Radioactive Ladies and Gentlemen,’ his letter began, ‘I have, with respect to … the continuous β-spectrum, hit upon a desperate remedy.’
Pauli’s suggestion was that β-decay produced, in addition to the observed electron, another heretofore unobserved particle. He called this particle the neutron, because it would have neither positive nor negative electric charge. On this model, the amount of energy released by each β-decay event as a whole would be, as expected, discrete. But as that energy could be split in a range of ways between the electron and Pauli’s new particle, the energy of the electron would take a continuous range of values.
This was a truly audacious posit. At the time, the only subatomic particles known to physicists were protons and electrons, and the idea of introducing an additional neutrally charged particle would have been, according to one historian of science, nothing short of ‘abhorrent’. Eugene Wigner, himself a purveyor of perplexing thought experiments in foundations of quantum theory, was reported to have said that his first reaction upon hearing of Pauli’s posit was that it was ‘crazy – but courageous’.
‘I admit,’ Pauli continued in his letter, ‘that my remedy may perhaps appear unlikely from the start, since one probably would long ago have seen the neutrons if they existed.’ The particle in question, he concluded, must have no charge and be very light. These characteristics would allow it to pass through material easily, and thus explain how it was able to evade detection. But what if it turned out that this new particle couldn’t be directly detected at all? In any case, he wrote, ‘nothing ventured, nothing gained.’ He then signed off his letter with apologies for not being in Tübingen in person – there was a ball he needed to attend in Zürich.
As far as historical anecdotes from physics go, this one is especially charming. It’s tempting to imagine Pauli composing the letter in his head while polishing his dancing shoes, reminding himself ‘nothing ventured, nothing gained’ as he headed out to his all-important dance. (One biographer surmises that it was the annual ball hosted by the Society of Italian Students in Zürich, and held at the Baur au Lac, the most distinguished hotel in the city.) Only days before, he had formalised his divorce following a short and ill-fated marriage to a German dancer, who had fallen in love with a chemist instead of her new husband. Both the profession and the mediocre standing of his romantic rival had frustrated Pauli. In one letter he wrote: ‘If it had been a bullfighter – with someone like that I could not have competed – but such an average chemist!’ At any rate, he clearly judged that taking concrete steps to move past his marital failure was more important than appearing in person in Tübingen to explore the possibility of a new particle, however crazy and courageous the latter might be.
Best of all, it turned out that Pauli’s desperate remedy was just the thing. A very small, very light, electrically neutral particle, which is emitted alongside an electron, does in fact explain the continuous β-spectrum. It isn’t the neutron – that name was commandeered by James Chadwick for the much larger neutral particle he discovered as part of the nucleus in 1932. But Pauli’s particle, which came to be called the neutrino, went on to gain widespread acceptance among physicists even though it would escape experimental confirmation until 1956.
As posits go, Pauli’s neutrino was an exceptionally good one. But that became clear only later
To this day, the neutrino remains one of the most mysterious subatomic particles. Tens of trillions of neutrinos from the Sun pass through our bodies every minute, and yet they interact so weakly with matter that physicists have to set up experiments deep in underground mine shafts or buried within the Antarctic ice to try to keep interfering signals to a minimum. The adjective ‘ghostly’ is widely used in popular science descriptions of the neutrino; one article in The New York Times, about the lengths physicists have to go to detect neutrinos, called them ‘aloof’. For a time,........
© Aeon


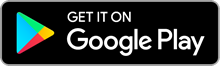